Learning objectives
- Describe how cells use different pigment molecules to absorb different wavelengths of light, and how this process relates to photosynthesis
- Compare and contrast photophosphorylation in chloroplasts to oxidative phosphorylation in mitochondria
- Identify the inputs and products of the light reactions of oxygenic photosynthesis
- Distinguish the capabilities of photosystem I versus photosystem II when in isolation versus together in the same organism
- Explain how life on Earth today is dependent on the evolutionary innovation of splitting water that led to oxygenic photosynthesis in cyanobacteria
- Identify the outcomes for electrons and proton gradients in cyclic versus non-cyclic (Z-scheme) electron flow, and differentiate between these two schemes
Photosynthesis creates organic carbon
Photosynthesis is the source of most organic carbon on Earth, as well as the oxygen in the air. The overall chemical equation for oxygenic photosynthesis is:
6 CO2 + 12 H2O –> C6H12O6 + 6 O2 + 6 H2O
We will present photosynthesis in two parts: this page will discuss the reactions that convert light energy to chemical energy in the form of ATP and reduced electron carriers (NADH or NADPH). Both are needed for carbon fixation reactions (the reduction of inorganic carbon to make organic carbon molecules) presented in the next page. An important by-product of the light reactions is the generation of oxygen gas. In the chemical equation above, the oxygen atoms in water are bolded in red to show that these are the source of the oxygen atoms in oxygen gas. Oxygenic photosynthesis evolved to take electrons from water to make oxygen gas, and ultimately give the electrons to carbon dioxide to form organic (reduced) carbon molecules (food) – the exact reverse of aerobic respiration, which takes electrons from organic carbon molecules and ultimately gives them to oxygen gas to make water.
Light reactions provide both ATP and Reducing Power (NADH or NADPH) needed for Carbon Fixation by the Calvin Cycle
There are two different general components of photosynthesis: the light reactions and the Calvin cycle. The light reactions require light (photons) and water as inputs, and they make ATP, NADH or NADPH, and oxygen. Oxygen is a waste product, but ATP and NADH/NADPH are essential for the next step, the Calvin cycle. The Calvin cycle is what actually fixes carbon (makes sugar), using the products of the light reactions (ATP, NADH/NADPH) and carbon dioxide. The product of the Calvin cycle is fixed carbon, or sugar.
In this section, we will focus on part one, the light reactions (require light; make ATP, NADH/NADPH, and oxygen). We will discuss part two, the Calvin cycle (requires products of the light reactions in terms of ATP and NADH/NADPH, to produce sugar from carbon dioxide), on the next page.
Light as energy
Visible light is a slice of the electromagnetic (EM) spectrum, from about 400 nm to a little over 700 nm in wavelength.
Light has wave-particle duality, and a quantum of light energy is a photon. The energy of a photon is inversely proportional to its wavelength: where h is Planck’s constant, c is the speed of light, and lambda is the wavelength. Therefore, photons of shorter wavelengths (blue-violet) have more energy than photons of longer wavelengths (red).
Biological pigments
Biological pigments are molecules that preferentially absorb light at particular wavelengths. Organisms that capture light energy for conversion to chemical energy show evolutionary and phylogenetic differences in the pigments they use.
Phototrophism vs photosynthesis
Phototrophic organisms convert light energy into chemical energy in the form of ATP. The use of light energy to make ATP is called photophosphorylation.
Photophosphorylation is similar to oxidative phophorylation in that both use a proton gradient across a membrane to power similar ATP synthase enzyme complexes. Photosynthetic organisms (photoautotrophs) use light energy to make both ATP and reduced electron carriers (NADH or NADPH). The reduction of carbon dioxide (CO2) to carbohydrate (CH2O) requires both ATP and reducing power in the form of NADPH or NADH (NADP+ is NAD+ with a phosphate group added, and is used by chloroplasts instead of NAD+). The earliest phototrophs and photosynthetic organisms were prokaryotes with single photosystems that did not generate oxygen. Two different types of photosystems evolved, that were combined in cyanobacteria. One of the two photosystems in cyanobacteria evolved the power to oxidize water molecules as a source of electrons, releasing O2.
Sidelight: Phototrophic Archaea
No Archaea thus found are truly photosynthetic. Halobacterium, which is an Archaeal species despite its name, uses bacteriorhodopsin, a purple-colored membrane protein, as a light-driven proton pump to generate a proton gradient across the plasma membrane and power chemiosmotic ATP synthesis. Thus Halobacteria are phototrophic, but not photosynthetic, because they do not use light energy to fix carbon dioxide to organic carbon. Phototrophic organisms still depend on organic food molecules to build their own biomass.
Photosynthetic bacteria
Photosynthetic bacteria and chloroplasts all use variants of chlorophyll.
Chlorophylls absorb blue and red light.
Here are two fantastic, quick videos about why chlorophyll is green:
Two types of photosystems
Photosystems are membrane complexes of proteins and chlorophyll molecules. The chlorophyll molecules absorb photons and funnel the energy to a reaction center chlorophyll, which becomes oxidized (loses electrons).
Cyanobacteria and chloroplasts are the photosynthesizers that produce oxygen as a by-product, and so are called oxygenic photosynthesizers. These all have two different types of photosystems coupled together. In contrast, all anoxygenic photosynthetic bacteria, the ones that do not produce oxygen gas, have just one type of photosystem. The earliest phototrophs were probably anoxygenic.
Photosystem I, when it becomes oxidized by absorbing light energy, transfers electrons to a protein called ferredoxin, which in turn reduces NADP+ to NADPH. NADP+ is an electron carrier, a phosphorylated form of NAD+; it may help to think of the P as standing for photosynthesis.
Photosystem II, upon absorbing light energy, transfers electrons to a membrane-localized electron transport chain, that pumps protons to generate an electrochemical gradient for chemiosmotic ATP synthesis. In cyanobacteria and chloroplasts, oxidized photosystem II splits (oxidizes) water molecules to regain electrons, and thereby generates oxygen gas.
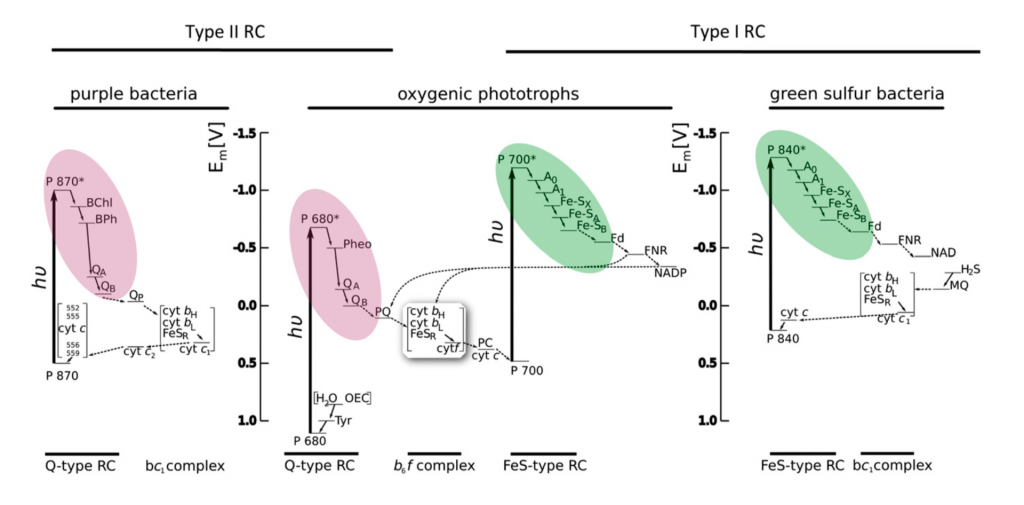
How did such a complicated system with two different photosystems evolve? Clues come from the observation that some phototrophic and photosynthetic bacteria have only one photosystem, and that single photosystem is either a type I or a type II photosystem.
Bacteria that have only a type II photosystem (PSII), such as the purple bacteria are phototropic: they use light energy to make ATP by photophosphorylation, but they do not use light energy to fix carbon dioxide. Photophosphorylation is very much like oxidative phosphorylation. In both, a membrane-localized ATP synthase complex uses power from a proton gradient to make ATP. Light energy oxidizes the reaction center chlorophyll and transfers electrons to an electron transport chain, which generates a proton gradient across the photosynthetic membrane. The terminal electron acceptors in purple bacteria are the oxidized reaction center chlorophylls; the electrons flow in a cycle from PSII down the ETC back to PSII. Purple sulfur bacteria with only PSII cannot use light energy to make NADH or NADPH, but they can use other pathways to oxidize hydrogen sulfide as a source of electrons to make reduced electron carriers. In other words, purple sulfur bacteria can fix carbon dioxide, but they cannot use their photosystem II to do it; their photosystem II can only be used to make ATP.
Bacteria with only a type I photosystem (PSI), such as green-sulfur bacteria, can be true photoautotrophs: they use light energy both to make ATP and to fix (reduce) carbon dioxide. Light energy oxidizes the reaction center chlorophyll, which reduces the the electron carrier NAD+ to make NADH. The oxidized reaction center chlorophyll must then be reduced by electrons from a chemical electron donor, such as hydrogen sulfide (H2S). The oxidized reaction center chlorophyll pulls electrons from H2S down the photosynthetic electron transport chain, which generates a proton gradient to make ATP. Thus green-sulfur bacteria use light energy to produce both ATP and reducing power; both are required for carbon fixation (reduction of CO2 to carbohydrate). However, they are limited by the availability of a suitable electron donor such as H2S.
Oxygenic photosynthesis and non-cyclic electron flow
Approximately 2.5-2.7 billion years ago, cyanobacteria evolved a scheme that coupled both types of photosystems. In the non-cyclic electron flow scheme (often called the Z-scheme), light-activated PSII gives its electrons to the electron transport chain to drive photophosphorylation. Simultaneously, light-activated PSI gives its electrons to reduce NADP+ to NADPH. The two systems are linked because the oxidized PSI is reduced by the electron transport chain (an electron is transferred from the ETC to PSI). Oxidized PSII regains electrons from oxidizing water molecules to generate oxygen gas. Therefore, in cyanobacteria (and chloroplasts), the flow of electrons is from water to PSII, then down the electron transport chain to PSI, and finally to NADP+ to make NADPH (cyanobacteria and chloroplasts use NADP+/NADPH instead of NAD+/NADH). The ability of cyanobacteria to extract electrons from water gave them a huge evolutionary advantage over green-sulfur bacteria, which were restricted to locations that had hydrogen sulfide or other suitable electron donors.
Photosynthesis in chloroplasts is essentially the same as photosynthesis in cyanobacteria. Both use non-cyclic electron flow to produce ATP and NADPH and O2. The figure below illustrates non-cyclic electron flow during photosynthesis in chloroplasts.

Cyclic electron flow in cyanobacteria and chloroplasts
When metabolic demands in the chloroplast require additional ATP, but no additional NADPH, cyclic electron flow from PSI through the electron transport chain and back to PSI can boost the proton gradient and thus photophosphorylation (light-driven ATP synthesis). Thus PSI is versatile in the sense that oxidized PSI can give electrons to either NADP+ (most of the time) or to the ETC (when needed to make additional ATP).
Photosynthetic membranes
The membranes of photosynthetic bacteria are highly convoluted via infolding of the plasma membrane, multiplying the surface area for light absorption and photosynthesis. These infolded membranes are are called thylakoids, and they are also present in chloroplasts, which evolved from endosymbiotic cyanobacteria. The lumen of the thylakoid corresponds to the extracellular or periplasmic space of the cyanobacteria.

This 5-min video gives a very nice animation of the light reactions of photosynthesis – students in Biological Principles do not need to remember the details of the electron transport chain nor the name of the enzyme that reduces NADP+ to NADPH.
And here is a short video lecture on the light reactions:
Powerpoint slides used for the video screencasts above: photosynthesis_lightreactions
References
Blankenship R.E., 2010. Early evolution of photosynthesis. Plant Physiol. 154:434-438. doi: 10.1104/pp.110.161687
Xiong J., W.M. Fischer, K. Inoue, M. Nakahara, C.E. Bauer, 2000. Molecular evidence for the early evolution of photosynthesis. Science 289:1724-1730. DOI: 10.1126/science.289.5485.1724
Sustainable Development Goal

UN Sustainable Development Goal (SDG) 13: Climate Action – Studying historic changes and biological innovations, such as oxygenic photosynthesis, that have lead to large-scale ecological changes can help us better understand organismal responses to climate change. By understanding how chemical processes are supported through the physiological properties of organisms, we can better predict which organisms might be most vulnerable to change and attempt to mitigate harmful effects, such as those of large-scale changes in gaseous resources.
Here’s a nice video animation of light reactions in a chloroplast: http://www.youtube.com/watch?v=hj_WKgnL6MI
Hello professor, i think this sentence is confusing. Shouldn’t the First “reduce” in this sentence be “oxidize”?
“Photosynthetic organisms also use light energy to reduce electron carriers (NADH or NADPH) required to reduce carbon dioxide (CO2) to carbohydrate (CH2O).”
I see how that might be confusing. It should be reduce NAD+ or NADP+ to NADH or NADPH. I’ll make the correction, thanks.
I have a question. In the section beginning with “Bacteria with a type I photosystem”, NAD+ and NADH are mentioned. Is that an error, or do these bacteria use NAD+ instead of NADP+?
These bacteria do use NAD+ and NADH. Cyanobacteria and chloroplasts use NADP+/NADPH
That is interesting. Thank you.
Hello Professor Choi 🙂 , I have a question on Phototrophism vs. Photosynthesis.
As I understood, phototrophic organisms generate ATP, but they do not reduce electron carriers such as NADP+, therfore there is no carbon fixation occuring.
Is that can also mean that Archaea that mostly metabolizes its energy through phototrophism, is Photoheterotroph? Because phototrophic organisms cannot fix carbon but still use light to generate energy? But I don’t see what complex carbon molecules that Archaea break down.
I refer flow chart from Wikipedia, and it classifies organisms into total four classes: photoautotrophs, heteroautotrophs, chemoautotrophs, and chemoheterotrophs.
And I have trouble with where to put phototrophism in which class.
Thank you 🙂
Youngmin Kim
Yes, the phototrophic Archaea depend on organic molecules as an electron source. They would be classified as photoheterotrophs. The chart from Wikipedia is incomplete. See http://en.wikipedia.org/wiki/Photoheterotroph
Can you explain the difference between phototrophs and photosynthetic organisms? I’m still a little confused.
Phototrophs use light energy to make ATP, but can’t do the carbon fixation part. Phototrophs still depend on organic carbon sources for their growth (make their lipids, carbohydrates, proteins, etc.). Photosynthesizers use light energy to fix carbon dioxide to make their own organic carbon.
Woah! I never really looked at photosynthesis/respiration in the way that you revealed to me. In the first intro video here, you discussed how cyanobacteria developed the method of accepting electrons from water, producing molecular oxygen. This led me to recall that carbohydrates are simply a storage molecule, which allows organisms to more-efficiently allocate energy over time.
This made me realize, evolutionarily, that all original organisms that utilized carbohydrates as a storage molecule would have produced carbohydrates themselves. This process of energy storage was for independent organisms. Photosynthesis (or ancestral carbohydrate-producing process) creates the storage while respiration uses the storage over time. This, of course, allows for greater specialization and complexity of organisms. (Here I disregard organisms that were able to accept free carbohydrate from the environment.)
However, then organisms evolved that were able to simply consume other organisms that already had a storage of carbohydrate, essentially stealing what the prey organism had worked hard for. This “stealing” or “consuming” process was even more efficient that the organism producing the storage molecule on its own, allowing for even greater organismal complexity. Humans, in fact, fall under this “stealing” category.
Pingback: In What Form Do Plants Store Energy? - UV Hero