Learning objectives
- Identify whether an organism is a heterotroph, photoautotroph or chemoautotroph based on their sources of energy and organic carbon
- Differentiate between substrate-level phosphorylation and oxidative phosphorylation
- Identify what molecule is oxidized, and what molecule is reduced in a redox reaction
- Explain the role of NAD+/NADH as an electron shuttle
- Explain how proton gradients are generated across membranes, and explain how ATP synthase exploits the proton motive force to make ATP
- Explain the relationships between cellular respiration, oxidative phosphorylation, chemiosmosis, and the electron transport chain
- Compare and contrast aerobic and anaerobic respiration
Types of cellular metabolism
The energy for ATP synthesis comes from organic molecules (such as carbohydrates), or from sunlight, or from inorganic electron donors. We can classify organisms according to their source of energy (used to make ATP) and their source of carbon (used to make organic compounds):
- chemoheterotrophs
- chemo: get energy from chemical reactions (oxidation of pre-existing organic compounds)
- hetero: get organic carbon from metabolism of pre-existing organic compounds
- examples include animals and fungi
- chemoautotrophs
- chemo: get energy from chemical reactions (oxidation of inorganic compounds) to make ATP
- auto: make their own organic carbon compounds from carbon dioxide
- examples include extremophiles (extreme-loving) bacteria or archaea that live in extreme environments like deep sea thermal vents or high-salt conditions like the Dead Sea (almost 10 times as salty as the ocean)
- photoheterotrophs
- photo: get energy from sunlight to make ATP
- hetero: get organic carbon from metabolism of pre-existing organic compounds
- examples include purple non-sulfur bacteria, green-non-sulfur bacteria, and heliobacteria
- photoautotrophs
- photo: get energy from sunlight to make ATP
- auto: make their own organic carbon compounds from carbon dioxide
- examples include cyanobacteria and green plants
Metabolic pathways carry out reactions that capture energy from these various sources (organic compounds, sunlight or chemicals) and couple them to synthesis of ATP from ADP.
Summary of cellular respiration essentials
- To make ATP, all a cell needs is a membrane, a gradient of protons across the membrane, a membrane-localized molecular machine called ATP synthase, and ADP and inorganic phosphate.
- The energy to power ATP synthesis comes from the proton gradient across the membrane.
- Heterotrophs, phototrophs and chemotrophs differ in the source of energy used to create and maintain the proton gradient – either chemical energy from organic molecules (heterotrophs), light energy (phototrophs), or chemical energy from inorganic molecules (chemotrophs).
- Regardless of the source of energy, the proton gradient is created by the flow of electrons down an electron transport chain*. Components of the electron transport chain couple electron transfers (oxidation-reduction reactions) to active transport of protons across the membrane, a process called chemiosmosis.
- In cellular respiration, the electrons for the electron transport chain come from NADH (which gets electrons from food molecules), and ultimately go to oxygen, or another terminal electron acceptor, to form water molecules.
- We define cellular respiration as the flow of electrons down the electron transport chain, and oxidative phosphorylation refers to the coupling of electron transfer reactions (oxidation-reduction reactions) to phosphorylation of ADP to make ATP.
*except in some phototrophic archaea that use bacteriorhodopsin to directly pump protons across the membrane using light energy—we’ll see that in the section on photosynthesis.
Introduction
The standard first-year biology textbook presentation focuses narrowly on glucose metabolism by animal cells, barely touches on fats and amino acids, and ignores most of the metabolic diversity of life. Moreover, the standard textbook version of how this elaborate metabolic network evolved beginning with glycolysis is probably wrong, accordingly to a compelling essay by Lane et al. (2010). In this class, we will take an evolutionary approach that begins with concepts and processes fundamental to all living cells that must have been present in the last universal common ancestor (LUCA).
Cellular Respiration: the BIG Picture
Before we get into any of the details, let’s start with a video and shows the big picture concept of how cellular respiration works and what it accomplishes (don’t worry yet about the narrator’s reference to other videos that explain these processes in detail; focus on the big picture only):
ATP synthesis
We find that all cells—Bacteria, Archaea, Eukarya—use the energy released via ATP hydrolysis (ATP –> ADP + Pi; Pi = inorganic phosphate; ΔG = -7.3 kcal/mol) to perform most of the cell’s work. How do cells make ATP? Cells can make ATP in either of two ways: either by substrate-level phosphorylation of ADP or by oxidative phosphorylation of ADP.
- ATP = adenosine triphosphate
- ADP = adenosine diphosphate
A substrate is a substance on which an enzyme acts. Substrate-level phosphorylation means that a phosphate is transferred from a high-energy phosphorylated organic compound (a substrate) to ADP. We will see in the section on metabolic pathways that a couple of the enzymes in glycolysis make ATP through substrate-level phosphorylation, as well as an enzyme in the citric acid cycle. However, only a small amount of ATP is made this way in cells undergoing respiration.

Oxidative phosphorylation refers to phosphorylation of ADP to ATP when coupled to oxidation-reduction reactions. It synthesizes the bulk of a cell’s ATP during cellular respiration. A proton-motive force, in the form of a large proton concentration difference across the membrane, provides the energy for the membrane-localized enzyme ATP synthase (a molecular machine) to make ATP from ADP and inorganic phosphate (Pi). The proton gradient is generated by a series of oxidation-reduction reactions carried out by protein complexes that make up an electron transport chain in the membrane. Those oxidation-reduction reactions gives their name to this process: oxidative phosphorylation.

The proton-motive force is a combination of a difference in proton (H+ ion) concentrations across a membrane, and the resulting electrical potential. All prokaryotic cells (Bacteria and Archaea) maintain a proton gradient (pH gradient) across their plasma membranes. Mitochondria maintain a proton gradient across the inner mitochondrial membrane. The interior of the bacterial cell (or the mitochondrial matrix) is relatively alkaline, whereas the exterior periplasmic space (or the mitochondrial intermembrane space) is relatively acidic. Because protons are positively charged, an imbalance of protons also creates an electrical charge difference across the membrane. This proton motive force is a form of stored energy, and protons returning across the membrane down their concentration and electrical charge gradients release free energy that can be harnessed by ATP synthase to make ATP. The lipid bilayer membrane is almost impermeable to protons, so the proton gradient is stable and normally does not discharge except via ATP synthase, or via proton channels that may open in the membrane.
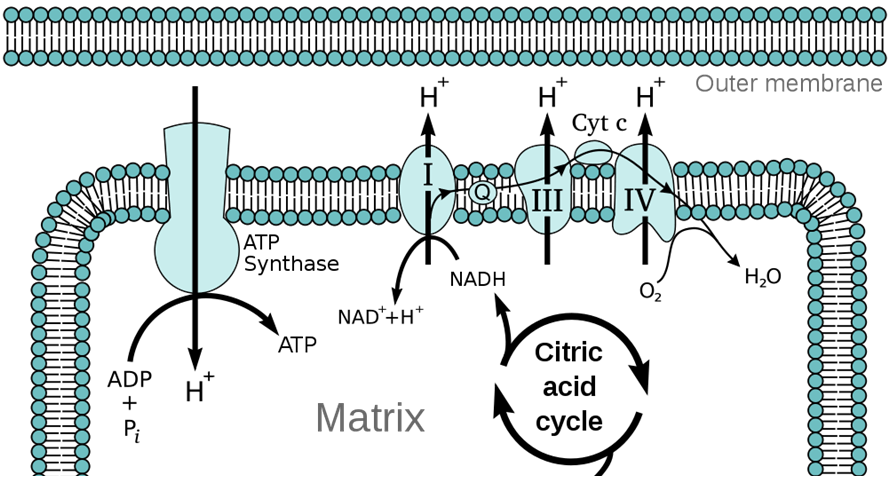
This proton gradient is analogous to water stored in an elevated reservoir. The higher the water level in the reservoir, the more potential energy is available to accomplish mechanical work like turning a water wheel to grind grain. In the same way, the greater the difference in proton concentrations across the membrane, the more energy is available for ATP synthase to make ATP. Indeed, the ATP synthase complex even resembles a water wheel, in that the flow of protons down their concentration gradient, through ATP synthase, causes a part of ATP synthase to rotate.
ATP synthase exploits the proton motive force to make ATP
The ATP synthase enzyme complex is located in the membrane, and is a remarkable molecular machine (Stock et al. 1999).
The proton motive force drives protons through a channel in the ATP synthase, and turns the rotor at approximately 100 rpm. The turning rotor changes the shape of the ATPase enzyme complex. The conformational change bonds ADP and inorganic phosphate together to form ATP. Each 360 degree turn of the rotor results in synthesis of 3 ATP molecules.
The ATP synthases in mitochondria, chloroplasts, and Bacteria are all structurally similar, and their amino acid sequence similarities are consistent with a common evolutionary origin (Watt et al. 2010). Lesser degrees of similarity, and more distant evolutionary relationships, exist with archaeal ATP synthases and a specialized organelles called a vacuole. Vacuolar ATPases pump protons across the membrane using the energy from ATP hydrolysis. Indeed, bacterial and mitochondrial ATP synthases can work in reverse to hydrolyze ATP and pump protons across the membrane to increase the membrane proton gradient (see end of video above).
What creates the proton gradient across the membrane? Chemiosmosis!
We have seen how ATP synthase acts like a proton-powered turbine and uses the energy released from the down-gradient flow of protons to synthesize ATP. The process of pumping protons across the membrane to generate the proton gradient is called chemiosmosis. Chemiosmosis is driven by the flow of electrons down the electron transport chain, a series of protein complexes in the membrane that forms an electron bucket brigade. Each of these protein complexes accepts and passes on electrons down the chain. For each electron they pass on, they pump a proton across the membrane. Ultimately, the last complex in the electron transport chain passes the electrons to a terminal electron acceptor. In aerobic respiration, this terminal electron acceptor is molecular oxygen (O2), and the reaction makes water.
We define respiration as the passage of electrons down the electron transport chain. We breathe (respire) oxygen because oxygen is the terminal electron acceptor, the end of the line for our mitochondrial electron transport chain. The video below shows the details of the electron transfer reactions and how they are coupled to pumping protons across the membrane. This is a form of active transport because the electron transfers release free energy that is used to pump protons against their concentration gradient.
Watch this video to understand how the ETC creates a proton gradient:
Here’s the video lecture explanation:
Many bacteria can use other terminal electron acceptors when oxygen is unavailable. These bacteria carry on anaerobic respiration, when the electron transport chain functions in the absence of oxygen, using an alternative terminal electron acceptor.
Redox Reactions and NAD+/NADH
The transfer of electrons from one molecule to another is called an oxidation-reduction, or redox reaction. A molecule that loses electrons is oxidized; a molecule that gains electrons is reduced. Different molecules have different tendencies to gain or lose electrons, called the redox potential. A redox reaction between a pair of molecules with a large difference in redox potential results in a large release of free energy.
In aqueous environments, the transferred electrons pick up protons. The result is that hydrogen atoms (a proton + electron = hydrogen) are transferred, and many enzymes that carry out redox reactions are called dehydrogenases. Living cells are the original hydrogen fuel cells.
Cellular energy metabolism features a series of redox reactions. Heterotrophs oxidize (take electrons from) organic molecules (food) and reduce (give them to) an electron carrier molecule, called NAD+ (in the oxidized form) that accepts electrons from food to become NADH (the reduced form). NADH then cycles back to NAD+ by giving electrons to (reducing) the first complex of the membrane electron transport chain. Thus NAD+/NADH is a key intermediary in shuttling electrons from food molecules to the electrons transport chain for respiration.
For more info, here’s a lecture snippet on NAD+ and NADH:
NADH is a high-energy molecule. The oxidation of NADH:
NADH + H+ + ½O2 –> NAD+ + H2O
is highly exergonic, with a standard free energy change of -54 kcal/mol (7-fold greater than the standard free energy change for ATP hydrolysis of -7.3 kcal/mol).
The membrane electron transport chain and chemiosmosis is a strategy for cells to maximize the amount of ATP they can make from the large amounts of free energy available in NADH. The electron transport chain subdivides the oxidation of NADH by O2 to a series of lower energy redox reactions, which are used to pump protons across the membrane. The resulting H+ concentration (pH) gradient across the membrane is a form of stored energy, analogous to an electric battery.
Anaerobic respiration in bacteria
The amount of energy released by these redox reactions, and thus the amount of energy available for ATP synthesis, depends on the redox potential of the terminal electron acceptor. Oxygen (O2) has the greatest redox potential, and thus aerobic respiration results in the most ATP synthesized. Bacteria and Archaea can use other terminal electron acceptors with lower redox potential when oxygen is not available. This anaerobic respiration produces less ATP.
Bacteria can modify their electron transport chains to use a variety of electron donors and electron acceptors, and will switch to the best available in their environment. In marine sediments, microbial communities stratify according to redox potential. The deeper, more anoxic layers use electron acceptors with progressively lower reducing potential.
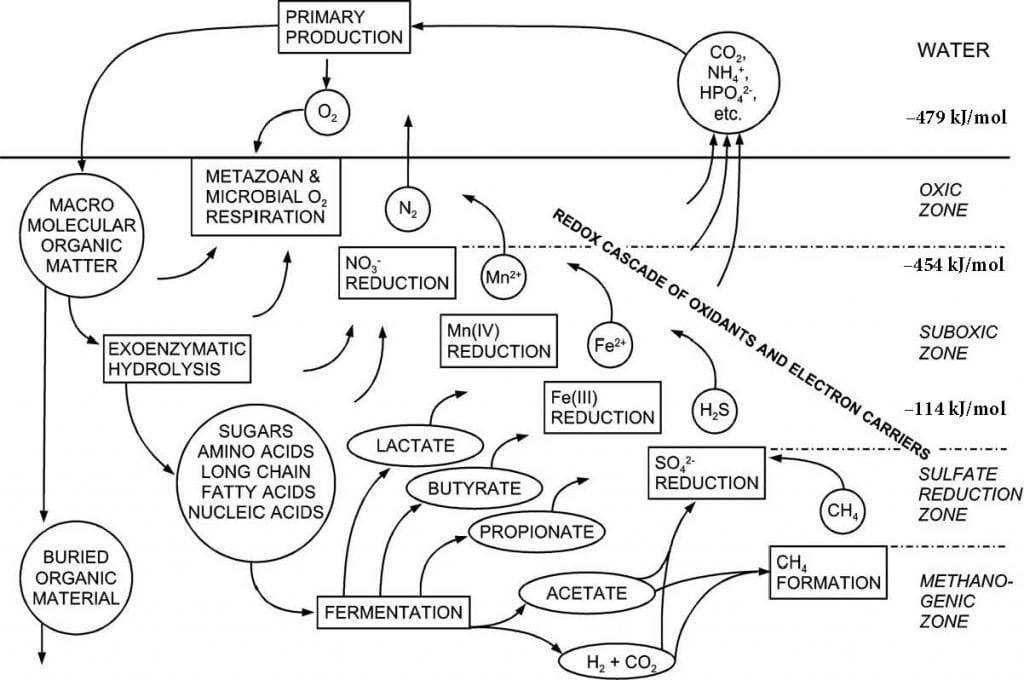
In addition to ATP synthesis, prokaryotic cells can use the proton motive force to supply energy for active transport of molecules across the plasma membrane, and to power the motor complex that rotates the bacterial flagellum.
This lecture video provides a generalized explanation of aerobic and anaerobic respiration:
An evolutionary perspective
The generation of a proton gradient across a membrane and chemiosmosis are universal to life on Earth and are fundamental ways for cells to make a living. Lane and colleagues (2009, 2010) speculate that “proton power” may have been the earliest form of energy metabolism, essential to, and pre-dating, the last universal common ancestor, LUCA.
The earliest cells, prokaryotes living in an early Earth devoid of free oxygen, used various alternative electron acceptors to carry on anaerobic cellular respiration. After cyanobacteria invented oxygenic photosynthesis and pumped oxygen gas into the oceans and atmosphere, bacteria that adapted their electron transport chains to exploit oxygen as the terminal electron acceptor gained higher energy yield and thus a competitive advantage. One line of aerobic bacteria took up an endosymbiotic relationship within a larger host cell, providing ATP in exchange for organic molecules. The endosymbiont was the evolutionary ancestor of mitochondria. This endosymbiosis must have occurred in the ancestor of all eukaryotes because all existing eukaryotes have mitochondria (Martin and Mentel 2010). The evidence for the endosymbiont origin of mitochondria can be found in:
- the double membrane of mitochondria, where the inner membrane contains the electron transport chain, derived from the plasma membrane of the aerobic bacterial endosymbiont
- the DNA of mitochondria, whose circular chromosome and genetic sequences resemble the alpha-proteobacteria.
- the ribosomes of mitochondria, which resemble prokaryotic ribosomes
Powered by hundreds or even thousands of mitochondria, eukaryotic cells attained larger sizes than their bacterial and archaeal predecessor, and eukaryotes evolved true multicellular lifestyles.
Put it all together
For reference, below is the full lecture video on this topic, which includes the 3 short segments excerpted above:
Powerpoint slides used in the video above: B1510_module3_5_respiration_2011Fall
A case study on microbial fuel cells to connect these ideas to real organisms in their environment.
References
Lane, N 2009 Was our oldest ancestor a proton-powered rock? New Scientist 19 October 2009 https://www.newscientist.com/article/mg20427306.200-was-our-oldest-ancestor-a-protonpowered-rock.html?page=1
Lane, N, JF Allen, W Martin 2010 How did LUCA make a living? Chemiosmosis in the origin of life. BioEssays DOI 10.1002/bies.200900131
Martin, W. & Mentel, M. (2010) The Origin of Mitochondria. Nature Education 3(9):58
Stock D, Leslie AGW, Walker JE 1999 Molecular architecture of the rotary motor in ATP synthase. Science 286:1700–1705. Abstract/FREE Full Text
Watt, IN, MG Montgomery, MJ Runswick, AGW Leslie, JE Walker 2010 Bioenergetic cost of making an adenosine triphosphate molecule in animal mitochondria PNAS 107 : 16823-16827 doi:10.1073/pnas.1012260107
Sustainable Development Goals


UN Sustainable Development Goal (SDG) 14 Life Below Water and SDG 15: Life on Land – Understanding the energy sources and carbon utilization strategies of different organisms can help us better conserve and manage ecosystems. It is important to identify types of energy and organic carbon processing (i.e., whether an organism is a heterotroph, photoautotroph, chemoautotroph, etc.) to understand an organism’s ecological role and how they contribute to the carbon cycle.
In the section of ATP synthesis, you mentioned how enzymes in the citric acid cycle can undergo substrate-level phosphorylation to produce small amounts of ATP, but the wikipedia article for citric acid cycle explains how the NADH generated by the citric acid cycle is fed into oxidative phosphorylation pathway in order to produce ATP. Does this mean that the citric acid cycle generates ATP by both substrate-level phosphorylation and oxidative phosphorylation?
Yes, the citric acid cycle makes a small amount of ATP by substrate-level phosphorylation, but the major energy product is reduced electron carriers, NADH and FADH2 (a molecule similar to NADH) that shuttle electrons to the electron transport chain for oxidative phosphorylation. This is explained in the next web page on oxidative pathways.
Revised 9/2016 to reorder LOs and some sections (begin with different types of cellular metabolism before diving into respiration), add summary of cellular respiration essentials, drop redundancies in evolution, cleaned up some typos and streamlined some explanations.
https://twitter.com/beatricebiology/status/839222378588594176/photo/1
The latest animation of E. coli F1 ATP synthase using structure data from cryo-electron microscopy:
https://static-movie-usa.glencoesoftware.com/mp4/10.7554/848/5aa66383dd7d1f479a50d4a6e4cd2e931d9423cb/elife-21598-media1.mp4